(image credit: Daein Ballard - CC BY-SA 3.0)
This article is part of a 2 part series on how existing companies can terraform Mars with current technology and a novel legal regime.
Hopes and dreams dashed?
New research appearing in a Nature article (*) indicates that it will be more difficult to Terraform Mars because of insufficient frozen Carbon Dioxide. News outlets all around the world took this article to mean that terraforming Mars now has proven to be impossible. But is it? And is the claim that we need novel technology correct?
As the authors put it in the abstract of their paper, they: “revisit the idea of ‘terraforming’ Mars — changing its environment to be more Earth-like in a way that would allow terrestrial life (possibly including humans) to survive without the need for life-support systems — in the context of what we know about Mars today. We want to answer the question of whether it is possible to mobilize gases present on Mars today in non-atmospheric reservoirs by emplacing them into the atmosphere, and increase the pressure and temperature so that plants or humans could survive at the surface. We ask whether this can be achieved considering realistic estimates of available volatiles, without the use of new technology that is well beyond today’s capability. Recent observations have been made of the loss of Mars’s atmosphere to space by the Mars Atmosphere and Volatile Evolution Mission probe and the Mars Express spacecraft, along with analyses of the abundance of carbon-bearing minerals and the occurrence of CO2 in polar ice from the Mars Reconnaissance Orbiter and the Mars Odyssey spacecraft. These results suggest that there is not enough CO2 remaining on Mars to provide significant greenhouse warming were the gas to be emplaced into the atmosphere; in addition, most of the CO2 gas in these reservoirs is not accessible and thus cannot be readily mobilized. As a result, we conclude that terraforming Mars is not possible using present-day technology.”
The authors looked at recent space mission analyses of the abundance of carbon-bearing minerals and the occurrence of CO2 in polar ice from the Mars Reconnaissance Orbiter and the Mars Odyssey spacecraft. And indeed, based on currently available data, the inventory they made of what is available on and below the Martian surface is not sufficient to turn the Martian atmosphere into one as thick as the one on Earth.
However, the claim that terraforming Mars is not possible with present-day technology is incorrect. Indeed, we can easily make the case that Mars can be terraformed in the time span it took the Dutch to rid their country of water, with currently existing technology.
Some factoids about the Martian atmosphere
The Martian atmosphere has between 0.65% and 1% of Earths atmospheric pressure (~101.315 Kilopascal). This means your blood would boil without a pressure suit at mere body fluid temperature. Not convenient and deadly. So today you do need a pressure garment and oxygen supply to perform activities at ground level.
Because the Martian atmosphere is mainly composed of C02 (98%), the authors looked at what a terrestrial pressure on Mars predominantly composed of CO2 would require. The extra C02 would also help with the Greenhouse effect, which must be made somewhat stronger at Mars than on Earth to compensate for the greater distance from the sun and to have it warm the planet on (almost) human timescales. C02 above a threshold is, of course, deadly to humans but if we succeed in warming Mars we will at the same time most likely make its gas composition more akin to the one on Earth. Still, the simplified exercise of only considering C02 is not meaningless as a first approximation to addressing the challenge. The current Martian atmosphere translates to 15g of CO2/m² or 6 mbar, compared to the main sea level pressure on Earth (EMSL) for which we would require 2500g CO2/m² or 1000 mbar (or one bar).
(On a side note, If you want some more background info about why Mars, in the period when it had running water, probably never solely was a CO2 atmosphere in the first place:
If you want even more in depth information, I suggest the article: “Technological Requirements for Terraforming Mars”, by Robert Zubrin from the Mars Society, who can give some basic outlines of the terraforming potential using resources available on Mars, which includes a look at other gases, and the use of easily producible orbital mirrors to get to 0.6 Bar.
However, according to the conclusion of the authors in the Jakowski article, using heating and energy-intensive strip mining approaches, Mars only has enough CO2 to locally create 15 millibars of pressure. This is still 65 times less than the pressure of 1,000 millibars at Earth’s main sea level (EMSL) and amounts to 1.5% of EMSL.
Nevertheless, the authors omit to notice that, elsewhere in their papers, they already deliver some very encouraging data points that will greatly benefit the Martians.
Can we produce EMSL pressure using locally sourced materials?
To produce EMSL pressure, the authors only considered local Martian polar CO2 ice or water-ice clathrate and CO2 adsorbed onto mineral grains in the regolith and carbon-bearing minerals (Martian carbonate-bearing rocks). The most accessible CO2 reservoir is in the polar caps. This CO2 ice could be readily mobilized by heating of the deposits. This could be done by, for example, using explosives to raise dust into the atmosphere so that it would deposit on the polar caps, effectively decreasing their surface albedo and increasing the amount of absorbed solar energy. Another way would be to use explosives or nukes to heat the ice, triggering sublimation. If the entire Mars polar-cap CO2 were to be gasified into the atmosphere, it would increase the pressure to less than 15 mbar total. Not enough.
Carbonate-bearing mineral deposits could also be heated to release their CO2. The typical breakdown temperature for carbonates is around ~300 °C. This is high enough that it could not be achieved by solar heating from greenhouse warming, and would thus require some form of processing. They limited such processing to the Nili Fossae soil deposits. Large-scale strip-mining here would probably put less than another 15 mbar and certainly no more than 150 mbar of CO2 into the atmosphere. Although other deposits that hold more CO2 exist, processing those would be more difficult due to either their more diffuse nature. Since deeper lying deposits cannot be located with the limited set of instruments in orbit, they were not a part of the studies.
Mars needs 65 millibars to reach the Armstrong limit (6.5% EMSL), ten times the current level. At higher pressures than this limit water no longer boils at body temperature. Notice that the optimistic estimate of 150 mbar in the Nili Fossae deposit is a multiple of the Armstrong Limit. Processing half of the Nili Fossae mineral deposits, in the quantities proposed by the authors of the paper, gets us to that amount.
The Martian atmosphere today has a mass of 25 Teratons (for 6 mbar) . We need to add at least another 200 Teratons (200 trillion tons) to reach the Armstrong limit at 65 mbar. Getting to Everest pressure would take about 1200-1400 trillion tons of Mars atmosphere. It will take about 4000 trillion tons to get to one earth main sea level pressure (EMSL).
Lower air pressure means there would be less warming. An atmosphere of 20 mbar would warm Mars with less than 10 K. Mars needs to be warmed with ~60 K to allow salty liquid water to be stable. It would take a CO2 pressure of about 1 bar to produce greenhouse warming that would bring temperatures close to the melting point of ice.
Indeed, when we only look at what is known to be available today, and using only the very rough data available today, we cannot get to EMSL, although in the most optimistic estimates (150 mbar) we are already 4 times above the Armstrong limit and could see humans walk around without a pressure suit, but with an oxygen mask. Furthermore, the heating and processing of the soil would require a lot of energy, which might not be the most efficient available approach. However, this is an energy requirement problem, not a technological one.
If you want your idea to make economic sense, you would like a faster and more practical approach.
But wait, there is a way that actually makes business sense: Venusian Imports.
Ready, aim, fire!
Venus has 92 BAR worth of CO2. That is 92 times the volume of Earths atmosphere, so it has plenty to share with Mars. It also has about 2% worth of Nitrogen, which can be turned into valuable fertilizer and/or buffer gas (Air on Earth is 21%O, 0.04% CO2 and 78% N2).
A smart entrepreneur would start importing gases from Venus to Mars, with a preference for N in order to produce Nitrogen and Ammonia for the growing agricultural needs of small colonies under domes. These needs are much lower and meeting them can show the world that the idea of transporting bulk goods from Venus to Mars makes economic sense.
Then again, 4000 Teratons worth of CO2 (for 1 bar EMSL), 4 kg with 18 zeros, shot from Venus with railguns has also become conceivable. Today BAE Naval railguns are at 2.6km/s and 32 Megajoule, but in a couple of decades we could conceivably shoot car sized shells of about one ton out of a barrel. Naturally, barrel technology is the showstopper in these technologies, since barrel life times become shorter the larger your projectile (and current density). However, mass drivers can trade strength of the materials used for length and a vacuum housing in a mostly vacuum environment. But let’s assume railguns with 2.6 km/s and be lenient on the masses we will be able to fire without shortening the lifespan of the barrel.
For comparison: The Germans in WOII used a siege artillary railway gun called “Schwerer Gustav” or “Heavy Gustaf” (and “Dora”).
Gustav was a 80 cm (31.5 in.) diameter gun developed to destroy the main fortifications of the French Maginot Line. The fully assembled gun weighed nearly 1,350 tonnes, and could fire shells weighing seven tonnes to a range of 47 kilometers (29 mi). It was indeed successful in destroying forts, but the 32m barrel needed to be replaced every 40 shots and could only fire about fourteen shots a day, due to its 45 minute reloading time. Given the wartime objectives this was an acceptable rate of fire and wear.
More recently, inspired by the successes in the long running SHARP project (Super High Altitude Research Project), a space gun project firing sensors on suborbital trajectories, a private company Quicklaunch, had been looking for about 500 M in funding to fire bulk materials to an orbiting depot using Hydrogen as the explosive to drive the light-gas gun (Mach 9 or about 3.063 km/s). The technology clearly worked but since they marketed their valid ideas too early, no investors appeared and the effort stopped in 2005 with a short revival in the 2010’s.”
Even if we need about 7.5 to 8 km/s to reach orbital speeds, the 2.6 km/s of a rail gun, including drag, is sufficient to reach a couple of hundreds of kilometers above the atmosphere, enough to be captured by orbital cantilevered systems (think of a high-tech trapeze-net that circles Venus and swings the payload it captures to a higher orbit). Reaching orbital speeds at low-cost with understood physics is pretty much guaranteed.
At an aggregation station in orbit, the contents of the shells will be added into larger packages, possibly in cubic volumes that can be hundreds of meters of even multiple kilometers on a side.
Next, we use thin-film Solar sails (mere micrometers thick) to drag the cargo to Mars.
For a first successful demonstration in this field, we can look at the JAXA IKAROS satellite, launched in 2010, which used the free solar wind and a 14m*14m solar sail to propel the 315 kg mass of the satellite (and only a 2 kg sail) from LEO all the way to Venus. We can thus safely extrapolate that larger sails will see us use free solar energy to send much larger masses in the opposite direction, from Venus to Mars. Since solar sails are cheap to produce, and we do not need to slow down, we can simply drop the package into the Martian gravity well where it will burn up, turn to gas and add to the Martian atmosphere. In a world growing accustomed to the practical implementation of automated drone-swarms on Earth (drone delivery networks) and in orbit (satellite constellations), the technological hurdles no longer exist.
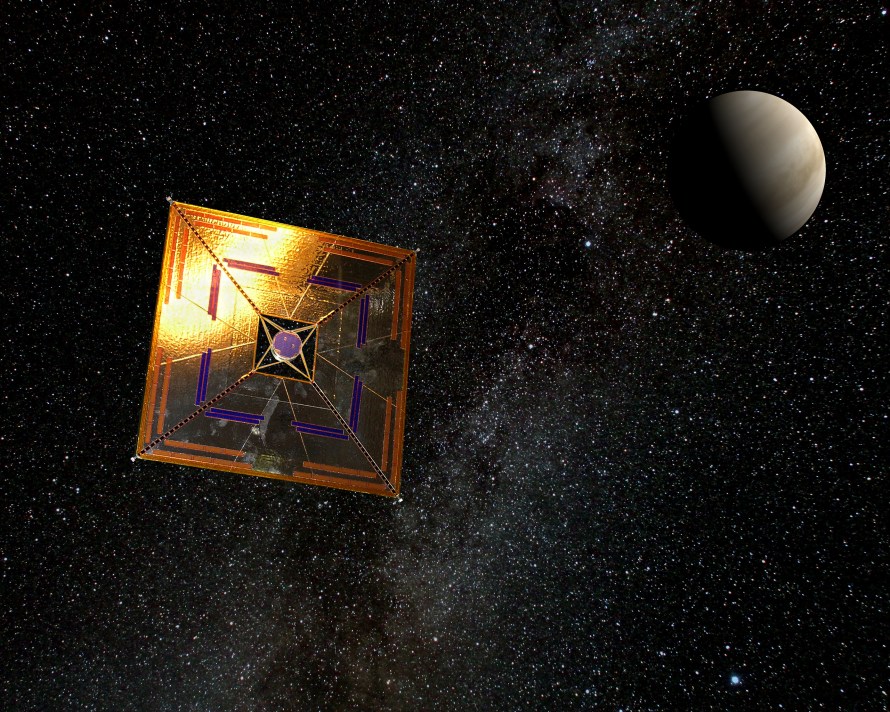
Contrary to what the authors present as a conclusion, currently available technology can already do the terraforming trick.
The back of the envelope calculation miracle
But what would be the numbers involved?
1000 Teratons of CO2 in kg, is 1 with 18 zeros. Thus for only 1 Tton / 1000 kg payload projectiles, 365 days, 24 hours, 60 minutes we get 1,902,588. Now… instead of once a minute, let us fire 10 times a minute, as in modern battle tanks. Now we arrive at 190,259. Number of rail guns: 1000…. We get 190 years. You can also increase the number of guns to use smaller shells within the range of fielded barrel technology. To be safe, we assume that lighter 100 kg projectiles will result in an unlimited barrel life, so our starting point to get 1 Teraton would be 10.000 rail guns firing 100 kg shells, 10 times per minute for 190 years.
190 years? That is the amount of time the Dutch needed to rid their country from water and become dry orange people, a business plan that I, as a Belgian, would have called highly imaginative, but not realistic. And I would have been wrong. Now, 10000 guns isn’t that many, compared to the number in the world inventory, or compared to the amount of over sized windmills China or Europe currently install every year. So… is it impossible? No, it sounds like the beginnings of a business plan.
But is this enough? And is it affordable?
Well. Not yet. We need about 4000 Teratons to have a thickness equal to Earths atmosphere, but we only need 250 Ttons to get to the Armstrong Limit.
The world has about 100.000 towed artillery pieces in active inventory, so the numbers involved, in a coordinated commercial effort, are not excessive. Using 100.000 pieces, we get to 10 Teratons in 190 years, combined with the 15 mbar present on Mars we now have almost half of the Armstrong limit. Continuing to use the by now ancient tech, we need 1000 more years to reach the Armstrong limit. Too long of an effort.
It is difficult to imagine that, once started, we will be using 1000 year old tech for a 1000 years and not invent a more efficient process or simply use larger guns, but let us first dig a little further in the business side of things using the railgun tech and see if we can reduce these timescales.
Today a rail gun is a prototype effort costing hundreds of millions in R&D. But within a decade it is conceivable that prices will drop to about 1 million per piece, certainly if the US NAVY wants to field more pieces on its ships, just as the Chinese are presently doing, and the British and Japanese are planning to do. We mustn’t over-exaggerate the complexity of rail guns and similar technology. A modern car is a much more complex item to design and manufacture and they are produced by the millions at low cost. Given mass production we will conservatively assume a high-ish 1 million dollar item price. Thus, for 1000 rail guns you would pay a billion, for 1 million pieces (which will probably results in a buyer discount) you pay 1 Trillion. That’s a lot of money.
A tale of two Apples
But wait a minute. Barring the option of coordinated government expenditures, … Apple, the private consumer electronics company, in 2nd quarter 2018 became the first company with a 1 Trillion dollar value in publicly traded shares. They would be able to pay for 1 million artillery pieces today. Maybe they can even call it the iGun and the iGun Pro (where i stands for interplanetary and Pro stands for… [“fill in your own joke”]). If they could convince their investors, and assuming no income growth, Apple alone would be able to give us 100 Teratons in 190 years.
The point is not to suggest that Apple should go into the railgun business, but that the idea that private companies can aggregate sums of money in the order of a Trillion or Trillions of dollars is no longer fictional.
Taking into account the 25 Ttons already present on Mars, this implies that two companies of equal Apple size would thus be able to get us to the Armstrong Limit within that time frame, give or take a decade. Want more pressure? Repeat or expand the operation. 12.8 companies the size of Apple, will be able to give us Everest pressure in 200 years. 40 companies the size of Apple -and why would we believe the average size of fortune 500 companies will get smaller in the future- will get us to 4000 Teratons, or EMSL pressure. 40 Apples would imply an installation cost of 40 Trillion dollar or 200 Billion dollars yearly for 200 years.
But what is 200 Billion dollar on a yearly basis? To our brains and emotions it still feels like an abstract amount of money, difficult to grasp. Let’s make it easier.
When we say Apple sized company we could substitute 40 business consortia or a group of Nations coming together to spend a mere 200 Billion per year for 200 years. There are currently 14 companies worldwide with a revenue of +200 Billion per year and we easily found 50 with more than 100 Billion in yearly revenue. (Some in this incomplete list are government owned enterprises, mainly in China, Russia and Japan). If you explore their diverse business areas, it becomes clear that you can make these sums of money by selling or trading a large range of commodities and not merely consumer goods. Most of these businesses are still growing. Apple, or a trillion dollar company, is not a pink unicorn that will remain unique. 200 Billion dollar, has become an example of the revenue more and more companies are making on a yearly basis.
(Source: good old https://en.wikipedia.org/wiki/List_of_largest_companies_by_revenue)
What 40 Apples can do
Great things happen when we put such sums of money to work in the task of terraforming a planet. In the case of Mars their combined efforts result in running water, plenty of Nitrogen for agriculture, plenty of C and O for humans, animals and plants. 1 Apple = more landed payload due to a thicker atmosphere. After 200 years: two Apples = no pressure suit, <14 Apples = Everest, 40 Apples = Eden on Mars. 40 Apples would also make sure that no pressure suits are needed after only a decade of operation [(200/(40/2) or 21.05 Teraton per year]. 14 Apples would achieve this Armstrong Limit within 30 years.
40 Apples and a 40 Trillion dollar installation cost? Feasible, check. Affordable? At 0.2 Trillion or 200 Billion/year it is affordable. Affordable, check. The last question will be to figure out if there is a Business plan that can generate 200 Billion in revenue on Mars each year for 200 years that includes shipping gases from Venus to Mars. Remember that there are already 14 companies in existence today on Earth that have figured out how to generate more than 200 Billion in yearly revenue. We will address the business case aspect in our next article (below).
In any case, if there existed a business plan that helped claim a tiny piece of land from the sea for 17 million orange people (the Dutch), than there is a business plan to reclaim a planet sized piece of land for…green people.
How does it compare to Global Warming reversal schemes?
Well one estimate, in the TED talk below, which uses a variety of measures puts the expenditure over 30 years at about USD 30 trillion (1 Trillion/Yr). In essence, we would be setting ourselves up to terraform our Terra/Earth. So our 40 Trillion over 200 years (200 Billion/YR) to terraform Mars, which has the same land mass as Earth, comes out favorably.
For comparison: the total cost of 100 solutions to reverse global warming
Where on Venus do we operate and get the materials for the guns?
Our base of operations for the rail guns is of course stationed on the roofs of balloons in the Venusian atmosphere. Normal breathable air is a buoyant gas in the CO2 atmosphere. Entire cities can drift in the clouds of Venus at 50 km high inside of these breathable air filled bags at EMSL temperatures. It is the stuff of science fiction but it works. Besides that, people will live in a 0.9 G , 1 atm of EMSL pressure environment. The Venusian atmosphere above will protect them from the Sun’s radiation, as good as at EMSL. For people who have their head in the clouds already, and like the sound of rail guns, Venus would be the ideal place to settle.
C02 has carbon in it, and Venus has 90 Earth atmospheres worth of CO2, plenty of carbon to make materials out of, including a wide range of plastics. MIT has already cracked the code on mass producing sheets of the miracle material graphene that can now be used as a conductor (replacing copper to create magnets), as a heat dissipation material, or as a structural material many times stronger than steel and lighter than plastic. Venus thus will become the ultimate carbon economy.
Rare materials can still be imported from Earth (or even the cold poles of hot Mercury, if you are willing to risk it) as mining the 400°C surface of Venus, could be an issue. That being said, and given time, some Venusian entrepreneurial spirit will probably find a way to mine the surface of Venus with long cables, high temp materials, or some other contraption.
Now we have to connect these rail guns, or similar tech, to a solar power supply. At Venus we can generate about double the solar power with terrestrial panels(**). Technology is in the works to triple solar panel efficiency to 60%(****), so we generate six times the energy of what we assume to be standard 21% efficiency today. But anything above factor two, is plenty. Any calculation using solar power can thus safely assume a six times smaller surface area than solar panel fields on Earth today. It would be an orbiting solar station, always illuminated and producing at max power 365/24/7. The excess heat produced by all these exposed surfaces can be dumped into the mined C02, the moment it leaves on the solar sails. This would present a novel approach to cooling spacecraft. (Spacecraft can normally only slowly radiate heat away since the much faster convection or conduction isn’t available in the vacuum of space, heating part of the mined C02 would solve this).
Martian Carbon Credits close the Business Case to Terraform Mars
in the next article, we will discuss a novel business case, next to agricultural nitrogen, to ship goods from Venus to Mars. The article is titled, “How the law will help in Terraforming Mars: Martian Carbon Credits (MCC) enable the Business Case”(**)
(*) Bruce M. Jakosky and Christopher S. Edwards, “Inventory of CO2 available for terraforming Mars”, NATURE ASTRONOMY, VOL 2, AUGUST 2018, pp 634–639, www.nature.com/natureastronomy, p. 634.
(**) Joris Luypaert, How the law will help in Terraforming Mars: Martian Carbon Credits, ONESTAGETOSPACE, (MCC)https://wordpress.com/post/onestagetospace.com/5759
(***)Above the Earth’s atmosphere the solar irradiance is slightly more than 1300 W/m2. At the Earth’s surface, with the Sun directly overhead at local noon (clear dry atmosphere), the solar irradiance is reduced to about 1000 W/m2 (1000 watts per square metre). At local noon on Mars, with Sun directly overhead, the solar irradiance is 590W/m2 (590 watts per square metre) (which, according to these 2 master thesis papers, is a value similar to what solar panels in scandinavia receive: Brekke, Tobias Aasprong, “Nordic irradiance conditions and the effects on solar module efficiency”, Master thesis, Norwegian University of Life Sciences, 2016-08-29, https://hdl.handle.net/11250/2402469, https://brage.bibsys.no/xmlui/handle/11250/2402469 : “The highest relative energy contribution occurs at 400-500 W/m2, contributing with 14 % of the yearly irradiation. The relative energy contribution is homogeneously distributed at low and medium intensities, but is drastically decreased after 800 W/m2. Irradiance levels ranging between 800-1100 W/m2 only contribute with 4 % of the yearly irradiation. The average relative efficiencies of the CIS- and p-Si modules at light intensities 400-500 W/m2, with respect to the STC efficiency of each module, are 0.9978 and 0.9987, respectively” and for more detail: Linda Hagen, MEASURED, MODELLED AND SATELLITE DERIVED SOLAR RADIATION IN SCANDINAVIA, MASTER’S THESIS IN METEOROLOGY, university of Bergen, 2011, https://bora.uib.no/bitstream/handle/1956/5149/84875326.pdf?sequence=1 )
(****) Adolfo De Sanctis, Iddo Amit, Steven P. Hepplestone, Monica F. Craciun & Saverio Russo, “Strain-engineered inverse charge-funnelling in layered semiconductors”,
Nature Communicationsvolume 9, Article number: 1652 (2018), 25 April 2018, DOI: 10.1038/s41467-018-04099-7, https://www.nature.com/articles/s41467-018-04099-7